Microbes of easy virtue
The genetic exchanges which have allowed bacteria to overcome antibiotics (11 minute read)
In the first year of the COVID-19 pandemic, which seems a very long time ago, I wrote about Wellington’s Bolton Street cemetery. While old cemeteries aren’t everyone’s thing, it’s actually one of my favourite places in central Wellington. It’s a peaceful refuge of soft grass and rustling leaves among the concrete and traffic noise, where daffodils smile in spring and old roses perfume the air in early summer.
I wrote about the cemetery because I was writing about the history of childhood mortality and vaccines, and there’s no better reminder of the dangers of childhood in years past than the sad graves where several children from the same family lie buried. I won’t repeat all the details from the previous article, but the short version is that for most of human history, around half of children didn’t reach adulthood. It’s a shocking figure and I’ve honestly got chills just writing this.
There were three innovations which allowed us to turn this around. One was better public and private hygiene, including hand washing and the treatment of sewage, resulting from our understanding of the role of microbes in disease. The second was the widespread use of routine childhood vaccination. The third was the discovery and use of antibiotics. Local circumstances, such as conflict or natural disaster, can mean that we aren’t able to use these three innovations in all circumstances. However, there is no reason to think that future generations will not be able to enjoy the benefits of better hygiene and vaccines.
The same cannot be said of antibiotics. This world-changing innovation is under serious threat. We are facing the prospect that future generations, already burdened by our failure to address climate change, will not be able to treat common infections.
You may wonder why I’ve taken an apparent diversion into public health, when I said just a few weeks ago that I was going to focus on topics such as genetic modification for the next few months. That is still my plan. In fact, I got some way into what I planned to be today’s article, when I realised I needed to take a step back. The history of genetic modification is tied up with our understanding of the way that certain types of living things genetically modify themselves. This self-modification is also part of the reason that antibiotics are losing their effectiveness. I realised that it made more sense to look at antibiotics and the remarkable ways that bacteria are managing to evade them, and then build on that understanding to explain how we harnessed that ability of bacteria.
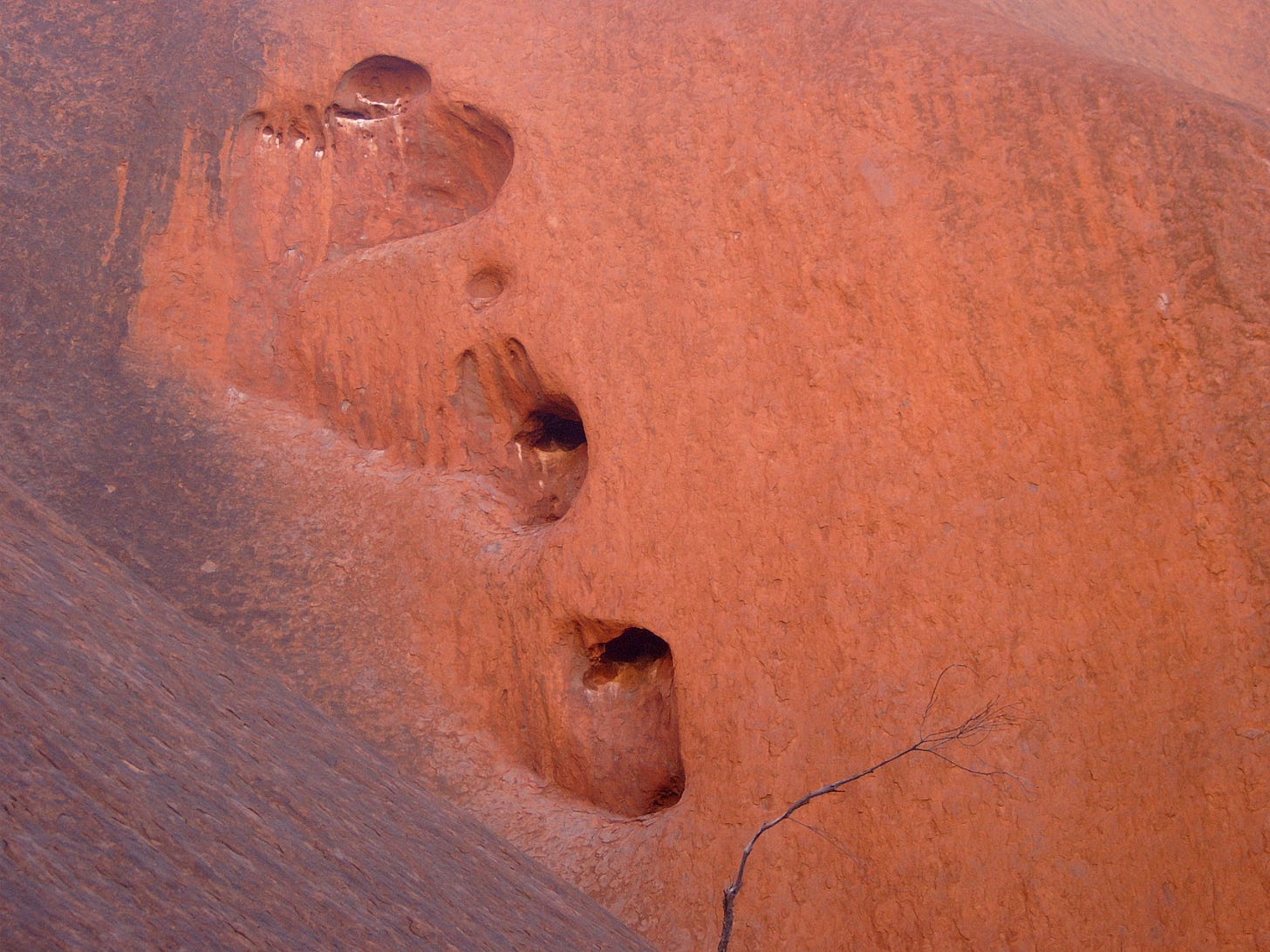
The history of antibiotics is often portrayed beginning with a moment of inspiration when Alexander Fleming discovered penicillin, but it’s far more complex than that. For a start, a number of ancient cultures recognised that applying mouldy bread could help in treating certain diseases. It’s hard to imagine how they discovered that, as wrapping up an infected wound with a piece of mouldy bread doesn’t seem like an intuitive thing to do. But when you consider what they used to treat diseases (leeches, anyone?), maybe it isn’t too unlikely.
In the early years of the 20th century, research conducted in Germany by a Polish Jewish doctor, a German chemist and a Japanese bacteriologist led to the discovery and development of salvarsan as a treatment for syphilis. Its introduction was little short of miraculous, since syphilis is a truly horrific disease. A more general treatment for bacterial infections came in the form of a drug called protonsil, which was discovered and developed by a German doctor in the 1930s. Both salvarsan and protonsil were developed from research into synthetic dyes.
The term antibiotic literally means against life, but it is most often used to refer to a compound which controls bacteria, and that is the way I’m using it here. Most of what we think of as antibiotics, such as penicillin, streptomycin, tetracycline and erythromycin, are produced naturally by soil-dwelling microbes. However, some are entirely synthetic, such as the sulfonamide or sulfa drugs, which were developed from protonsil (salvarsan was a synthetic arsenic compound, dangerous and unstable, but still better than syphilis). Different antibiotics affect bacteria in different ways – some affect the wall which surrounds the bacterial cells, others affect various processes inside the cell. Some kill the bacteria, but others simply inhibit their growth.
In the early 1940s, well before penicillin came into widespread use, reports began to emerge of bacteria becoming resistant to the drug. There are a number of different explanations for why this happens – all are basically correct, but they frame the problem in different ways. The most common explanation is that we have overused and misused antibiotics, and this is why resistance has developed. While this is correct, it doesn’t really explain the process.
A more detailed explanation comes from Darwin’s description of natural selection. In a population of living things, whether humans, dogs or bacteria, there will be variation in all kinds of traits. This could be their appearance, their size or it could be how they respond to diseases and toxins. This is true for any trait influenced by genes, whether it is the ability to herd sheep, to shake off an infection of COVID-19 or survive being sprayed with DDT. This also applies to a population of bacteria, for example those in an infected wound – some will die at the merest sniff of penicillin, while others will barely flinch, metaphorically speaking. So, when a wound has been treated with penicillin, the susceptible bacteria will all be gone, and the only ones left to reproduce will be the ones which can cope with the drug.
But where does that variation come from? This is where things get weird. The curious thing about bacteria is that they mostly reproduce by simply dividing in two. The don’t reproduce sexually in the way that animals and plants do. There’s no process of mixing up the genes, so that the offspring end up with a unique combination of genes and therefore a unique set of characteristics.
The genes of bacteria are different from the genes of humans, dogs, pine trees and all other plants and animals, as well as fungi and seaweed. Some months ago, I wrote about the three basic divisions of living things. Animals, plants, fungi, seaweed and a few other weird and wonderful groups most people have never heard of all belong to a single group. All of these have linear DNA – basically the DNA molecules are like long threads, known as chromosomes. Different species have different numbers of chromosomes, which are always paired.
Bacteria have only one chromosome, or molecule of DNA, and it is circular. When a bacterial cell reproduces, it simply copies this single chromosome and then the cell splits in two with one copy of the chromosome going to each new cell. It’s a much less complicated process than dividing the cell of a human, or even something like yeast. Because it’s less complicated, it’s usually faster as well.
Variation happens in two ways. The first is simply a copying error. The information in DNA is encoded in the order of four different components represented by the letters A, C, G, and T (I’ve explained this in more detail in the article here). Sometimes the cell slips up in the copying process and replaces one letter with another. This means that the resulting offspring has slightly different genes from the parent and this may result in different traits. Mostly, these differences provide no benefit, or are harmful. But, every now and again, there’s a difference which provides a benefit. The cells with this difference grow a little bit better than cells without the difference, and eventually come to dominate.
This is the basic process of natural selection as described by Darwin, and on the surface it appears to explain how bacteria became resistant to antibiotics. In theory, if one of these differences allows bacteria to better tolerate a certain kind of antibiotic, then the use of that antibiotic will allow those bacteria to compete more effectively with other bacteria. But this isn’t actually what has happened. The basis of evolution, as described by Darwin, is competition. The way that numerous kinds of bacteria all around the world have become resistant to antibiotics in little more than half a century is something else entirely. Bacteria cooperate – not just within, but also between, species.
Bacteria don’t have sexual reproduction as we think of it in animals and plants. Nonetheless, there are many different ways that bacteria share DNA, and they do it freely, not just within a species but between species. They do it so freely that researchers writing in academic journals sometimes get away with describing bacterial DNA exchange as promiscuous. These ways of exchanging DNA are crucial to understanding both antibiotic resistance and some types of genetic modification. For now, I’m only going to look at one way, but the principles for all are similar.
As well as having their single chromosome, bacteria can contain small pieces of DNA known as plasmids (like the chromosome, these are also circular). Plasmids contain only a few genes, sometimes only one. They can be passed from one bacterial cell to another. They can also exist in the environment outside a bacteria cell, and be picked up by other bacteria.
Reproducing plasmids takes energy for the bacteria which have them, but it’s worth their while, because they help the bacteria survive stress in their environment. For example, Listeria bacteria, which cause food poisoning and are particularly dangerous to pregnant women, have plasmids which allow them to tolerate higher levels of acid, disinfectant and heat, all useful abilities in a microbe making its home in our food.
Sources of stress can include antibiotics, which are, after all, mostly chemicals which bacteria could encounter in the natural environment. So, if an antibiotic is used against an infection in a person, and there are bacteria in that person’s body with plasmids for resistance to that antibiotic, then what happens next is exactly what you’d expect. The bacteria share the plasmid, and suddenly all kinds of bacteria, not just the type being targeted, are resistant. In fact, it can be even worse than that. The bacteria may even share the resistance plasmid in the absence of the antibiotic, so the first time it is used with that person, it doesn’t work.
When we began using antibiotics on a large scale, plasmids for resistance suddenly became very useful to bacteria. And instead of hoarding those plasmids so that they could become dominant, the bacteria which had them shared them around, giving their neighbours, even unrelated species, a helping hand. Okay, I’m anthropomorphising slightly, but you get the picture.
So, at the level of individual bacteria, that is how antibiotic resistance developed and spread. Zooming back out to look at the wider picture – the problem is more than just prescribing antibiotics to people who don’t have bacterial infections, although that’s part of it. Studies have found that a substantial proportion of the antibiotics prescribed are inappropriate – the wrong antibiotic, the wrong dose or even simply not necessary. There has also been the widespread use of antibiotics as growth promoters in livestock. How much this kind of antibiotic use contributed to resistance was debated for many years, but it has become increasingly clear that it is part of the problem. There are other ways we have spread antibiotics around in the environment as well. Antibiotics pass through our bodies largely intact, meaning that wastewater, especially that coming from places like hospitals, now contains antibiotics. Even treated wastewater still contains antibiotics, and contributes to the resistance problem.
Can’t we just find new antibiotics? Maybe. Some sources are optimistic about our ability to discover new antibiotics, and new ways to treat infection. Others are less so, pointing to the fact that pharmaceutical companies are not particularly interested in developing them, and public good research funding keeps getting cut. The point about the interest of pharmaceutical companies is an important one. If you were running a company, would you put your research budget into vaccines, which stop people from getting sick, or antibiotics, which cure them quickly? Or would you try and develop something like semaglutide which treats chronic health problems in rich countries and largely stops working once people stop taking it? I think we all know the answer.
From what I’ve read, the problem of antibiotic resistance is rather like climate change. Developing new technologies is important, and it won’t happen if we leave it entirely in the hands of private companies. We – and I mean taxpayers and governments – must support research for the public good. But, just as we can’t address the climate change without making drastic cuts to our fossil fuel use, we can’t solve antibiotic resistance without making major changes to how, and how often, we use these lifesaving medicines.